All reagents and solvents were purchased from Sigma-Aldrich and were used as received. Bacterial strains were purchased from the American Type Culture Collection.
Hydrazone Schiff base ligands were prepared by directly mixing an equimolar ratio (1:1) of either benzyhydrazide, isonicotinic acid hydrazide (isoniazid) or 4-phenylsemicarbazide with cinnamaldehyde (Scheme 1) in 20 mL of hot acetonitrile and heated under reflux at 60 °C for 2 h, affording N′-((1E,2E)-3-phenylallylidene)benzohydrazide (L1), N′-((1E,2E)-3-phenylallylidene)isonicotinohydrazide (L2), and (E)-N-phenyl-2-((E)-3-phenylallylidene)hydrazidecarboxamide (L3), respectively. The hydrazone compounds formed are poorly soluble and they precipitate out as solids overnight in cold temperature (4 °C). The yellowish precipitates are easily separable through filtration and the compounds were washed with cold acetonitrile and subsequently dried at room temperature. The purity for all compounds was inspected using 1H and 13C-NMR along with melting point measurement. Attempts to crystallise the hydrazone Schiff base ligands for singlecrystal diffraction study were not successful. The chemical shifts for L1, L2, and L3 are seen as follows:
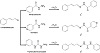
Scheme 1: Synthesis of L1, L2, and L3 from cinnamaldehyde and three different hydrazides.
L1: 1H-NMR (400 MHz, DMSO-d6) δ ppm: 11.73 (s, 1H, N-H), 8.20 (d, 1H, C-H), 7.86 (d, 2H, Ar-H), 7.60 (d, 2H, Ar-H), 7.55 (d, 1H, C-H), 7.49 (t, 2H, Ar-H), 7.37 (t, 2H, Ar-H), 7.30 (t, 1H, Ar-H), 7.04 (m, 2H, Ar-H; C-H); 13C-NMR (400 MHz, DMSO-d6) δ ppm: 163.54, 150.30, 139.60, 136.44, 133.92, 132.29, 129.39, 129.02, 128.15, 127.65, 126.20; IR: ν(cm−1) = 3,267 (m, NH), 1646 (s, C=O), 1,487 (m, C=N); Tm = 199–202 °C.
L2: 1H-NMR (400 MHz, DMSO-d6) δ ppm: 11.94 (s, 1H, N-H), 8.75 (d, 2H, Ar-H), 8.21 (d, 1H, C-H), 7.78 (d, 2H, Ar-H), 7.61 (d, 2H, Ar-H), 7.38-7.30 (m, 3H, Ar-H), 7.07 (m, 2H, C-H); 13C (400 MHz, DMSO-d6) δ ppm: 162.01, 151.52, 150.86, 140.97, 140.50, 136.31, 129.40, 127.76, 125.90, 122.07; IR: ν(cm−1) = 3,243 (m, NH), 1,651 (s, C=O), 1,491 (m, C=N); Tm = 198–201 °C.
L3: 1H-NMR (400 MHz, DMSO-d6) δ ppm: 10.66 (s, 1H, N-H), 8.76 (s, 1H, N-H), 7.75 (d, 1H, C-H), 7.60-7.51 (m, 4H, Ar-H), 7.38-7.22 (m, 6H, Ar-H), 6.95 (m, 2H, C-H, Ar-H); 13C-NMR (400 MHz, DMSO-d6) δ ppm: 153.35, 143.46, 139.63, 137.61, 136.60, 129.43, 129.08, 127.29, 125.98, 122.86, 119.81; IR: ν(cm−1) = 3,341 (m, NH), 1,670 (s, C=O), 1,445 (m, C=N); Tm = 175–177 °C.