The full characterization of H2LA2 and H2LA3 are reported elsewhere [17,18], whereas H2LA1 and its corresponding Cr-complex (CrLA1) are studied and discussed here in this work.
Cr(III)-imine complexes (CrLA1, CrLA2, and CrLA3) are tinted in solid phase at 25 °C and nonhygroscopic in nature. The elemental analysis results of H2LA2, H2LA3, and their Cr(III)-complexes are presented elsewhere [17,18]. The elemental analysis results of CrLA1 and its coordinated ligand H2LA1 are listed in Table 1, which are convenient with the theoretical percentages of CHN elements. Consequently, H2LA1 behaved as coordinated chelating agent towards Cr(III) ion as tri-dentate in 1 : 1 ratios to Cr3+ ion in an octahedral geometry, similar to CrLA2. The molar conductance of CrLA1 was measured at 25 °C in DMF with 7.02 Ω−1 cm2 mol−1, as summarized in Table 1. The conductivity value implies the nonelectrolytic nature of CrLA1 and is convenient with the suggestion that the NO3− anion is in the coordination sphere (Scheme 1). Magnetic moment measurements of CrLA2 and CrLA3 are recoded elsewhere [17,18], affording paramagnetic properties, but for CrLA1, it is reported in Table 1. The paramagnetic feature of CrLA1 is recorded with magnitude 4.08 BM.
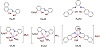
Scheme 1: Molecular structure of H2LA1, H2LA2, and H2LA3 ligands, and their Cr(III)-complexes (CrLA1, CrLA2, and CrLA3).
1H- and 13C-NMR spectra of H2LA2 and H2LA3 are already presented previously [17, 18]. The 1H- and 13CNMR spectral scans and their data of H2LA1 are listed in Section 2. The most characteristic 1H-NMR spectrum of H2LA1 showed a singlet signal at 15.65 of naphthyl-OH, at 10.15 of phenyl-OH, and 9.49 ppm of azomethine group (CH=N). The rest signals belong to the aromatic protons of phenyl and naphthyl rings in H2LA1.
The most distinguished 13C-NMR signal is at 177.1 ppm as CH signal of H2LA1, which is assigned to azomethine carbon. The other signals, detected in the region 150.4-10.3 ppm, are exhibited for the phenyl and naphthyl carbon atoms.
The featured IR wave number data of the H2LA2, H2LA3,
and their complexes (CrLA2 and CrLA3) are reported [17, 18]. For H2LA1 and CrLA1, the most significant vibrational bands are tabulated in Table 2. The −OH and −CH=N− groups are assigned with broad and sharp bands in H2LA1 at 3,448 cm−1 and 1,634 cm−1, respectively. With complex formation with Cr3+ ion, the −OH band could not be observed anymore and the −CH=N− band was notably low shifted to 1,583 cm−1. That lower shifting after CrLA1 formation of the azomethine nitrogen atoms could prove the role of nitrogen lone pair of azomethine group to bond to Cr3+ ion within coordinated bond [23]. An additional band was detected at 3,378 cm−1 due to the appearance of the coordinated water molecules or the crystalline molecules. This suggestion could be supported by the presence of new stretching weak bands at 567 cm−1 and 460 cm−1, corresponding to the Cr−O and Cr−N bonding formation (Table 1) [23,24]. The distinguished frequencies of NO3− group in CrLA1 have three nondegenerated modes at 1,470 cm−1 υ(NO2)asy, 1,356 cm−1 υ(NO2)sy, and 851 cm−1 υ(NO) [25].
The molecular electronic transition spectral measurements of H2LA2, H2LA3, CrLA2, and CrLA3 were recorded and discussed before [17,18]. Here we presented only the characteristic electronic absorption transitions of H2LA1 and its Cr-complex (CrLA1) to utilize their stereochemistry according to the sites and number of possible electronic transition bands [16]. H2LA1 shows two registered bands at 355 nm and 496 nm for the n→π* and L-CT transitions in the visible regions [22, 26]. CrLA1 assigned three bands at 338 nm, 404 nm, and 450 nm, which are resulted from n→π* and ML-CT, and d–d transitions. The band d–d could be mainly proven by the formed Cr-complex (Figure 1).
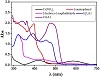
Figure 1: The electronic spectra of reagents and their synthesized H2LA1 and its corresponded complex (CrLA1).
The thermal behavior of CrLA2 and CrLA3 was presented previously [17, 18]. But CrLA1 was analyzed thermally and the results were illustrated in Table 3. In the table, the hydrated CrLA1 in the crystal lattice lost two H2O molecules in the first step. That mass loss was 8.12%, which agrees with the calculated values 8.05%. The second loss is the two H2O coordinated molecules with decomposing of the coordinated ligand molecule in the next steps, as noted in Table 3. The experimental percentage loss is 8.22%, which is also convenient with the theoretical one, 8.05%. The TGA curve of CrLA1 shows loss in weight within the temperature range 213–285 °C, which is attributed to the removal of the coordinated nitrate anion (found 13.87% and calc. 13.78%). Furthermore, CrLA1 assigned mass loss in the temperature range 287–399 °C, which is resulted from the loss of an organic part of the coordinated ligand (C8H8O2) with mass losses (found 31.65% and calc. 31.77%). The last decomposition step was done for the organic species at the range of temperature 401–567 °C with mass losses (found 26.52%, calc. 26.60%), leading to the loss of the last species of the coordinated ligand. At the end, formation of free Cr atoms as the final product was discovered at temperature more than 567 °C (found 11.70%, calc. 11.63%) [23,24].
Kinetic parameters are displayed in Table 3. The value of G* was increased by temperature arising. The H* was recorded as a positive value referring to endothermic decomposition processes of CrLA1. Moreover, the negative value of S* suggested a decomposition via abnormal pathway at the above decomposed steps. The negative activation entropy proposes a high order of the formed complex CrLA1 than that of the substrates and so the formation reactions are considered to be slow. Furthermore, the positive values of H* and G* (Table 3) suggested endothermic features for each thermal step [23,24,27].
Stoichiometry of CrLA2 and CrLA3 is determined previously [17, 18]. For CrAL1, its stoichiometry was determined within two various methods spectrophotometrically; first is the continuous-variations method and second is the mole-ratio method. The obtained results showed that the stoichiometry of CrLA1 is 1 : 1 [22, 26].
The formation constants (Kf) of CrLA2 and CrLA3 are reported and presented elsewhere [17,18]. Here Kf value was evaluated only for CrLA1 depending upon the spectrophotometric measures of the continuous variation method. The obtained Kf value displays a high stability of CrLA1. In addition, stability constant (pK) and Gibbs free energy (ΔG≠) values of CrLA1 were derived and calculated
(Table 3). The negative value of Gibb’s free energy means that CrLA1 formation reaction of H2LA1 and Cr3+ ion is spontaneous and favored.
The catalytic reactivity of the three Cr(III)-imine complexes was investigated in the oxidation of benzyl alcohol (R) as a standard model of primary alcohols with an aqueous H2O2,
as an oxygen donor, to the corresponding carbonyl compound, BA, in various aerobic conditions. A series of blank tests revealed that the presence of CrIII-catalyst and H2O2, as the oxidant, is essential for an effective catalytic oxidation of benzyl alcohol (Tables 4, 5, and 6). In order to find the optimized reaction conditions, the effect of alternative reaction parameters was studied that may influence the control chemoselective conversion to BA. Temperature, time, and solvent are the most fundamental factors that have been examined to get the optimized catalytic processes.
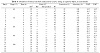
Table 4: Oxidation of benzyl alcohol catalyzed by CrLA1 using an aqueous H2O2 in acetonitrile.
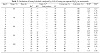
Table 5: Oxidation of benzyl alcohol catalyzed by CrLA2 using an aqueous H2O2 in acetonitrile.
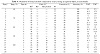
Table 6: Oxidation of benzyl alcohol catalyzed by CrLA3 using an aqueous H2O2 in acetonitrile.
So far in literature, catalytic studies on Cr(III) complexes are reported for the oxidation of alcohols or (ep)oxidation of alkenes and alkanes [11, 28]. Some attempts have been published for the investigation of the catalytic potentials of some Cr(III)-complexes supported on silica gel [29] or MCM-41 [30]. It is a new attempt to present here the catalytic activity of new Cr(III) complexes of tri- and tetradentate imine ligands with different moiety and bulkiness.
The benzyl alcohol (R) (1.0 mmol) oxidation processes are carried out with an aqueous H2O2 (3.0 mmol) in acetonitrile (10 mL) at various temperatures and for different times catalyzed by CrLA1, CrLA2 or CrLA3 with various catalytic molar ratios of the catalysts (0.02 mmol, 0.05 mmol or 0.10 mmol); as shown in Tables 4, 5, 6, and 7. The obtained oxide products could be analyzed and identified by GC. Controlling of each experiment showed that no oxide product (P) could be formed in the absence of Cr(III)-catalyst.
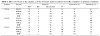
Table 7: Effect of solvent on the catalytic activity of benzyl alcohol oxidation by Cr(II) complexes at optimum conditions.
The controlled catalytic processes using CrLA1, CrLA2 or CrLA3 catalyst were entirely chemoselective for oxidation. BA, as the major target product, was obtained in the highest scale, but there were other unwelcomed side products in alternative conditions, as obtained by the turnover numbers (TON) and turnover frequencies (TOF) (Tables 4, 5, and 6).
Depending upon temperature control, at 60 °C, the conversion of A to BA catalyzed by CrLA1, CrLA2 or CrLA3 was very low, but the chemoselectivity was an excellent approach to 100% as observed in Tables 4, 5, and 6 (entries 1 and 2) for all catalysts after 4 h. But by time, the selectivity was reduced for all catalyst complexes after 6 h to be 71%, 61%, and 60% with CrLA1, CrLA2, and CrLA3, respectively, see entry 4 in Tables 4, 5, and 6. At 60 °C, the reaction temperature is not enough for improving the catalytic potential of the studied Cr(III)-catalysts.
By increasing of the reaction temperature to 70 °C, the conversion was improved unremarkably in the identical conditions (Tables 4, 5, and 6, entries 5 and 6), and the selectivity was excellent (100%), affording good yield of BA (47%, 44%, and 40% catalyzed by CrLA1, CrLA2, and CrLA3, resp.) after 2 h with no observation of BZ as a further oxidized product. With time running, the percentages of BA were enhanced with time after 4 h to 52%, 49%, and 43% by CrLA1, CrLA2, and CrLA3, respectively, with observation of other unknown side products percentages (Tables 4, 5, and 6, entry 7), along with an increase of conversion and a decrease of the control selectivity. Furthermore, after 6 h at the same temperature (70 °C), the chemoslectivity was reduced continuously to be 87%, 82%, and 85% of BA with CrLA1, CrLA2, and CrLA3, respectively, as recorded in Tables 4, 5, and 6, entry 8. We can deduce here that the catalytic oxidation of benzyl alcohol by an aqueous H2O2 is catalyzed by CrLA1, CrLA2 or CrLA3 influenced by temperature.
Nevertheless, at 80 °C, both the selectivity and the conversion percentages were highly observable, enhanced, and progressed up to 93%, 85%, and 74% of BA with optimized chemoselectivity (100%) (i.e., no presence of BZ or other unknown side products for CrLA1, CrLA2 or CrLA3, resp., as shown in Tables 4, 5, and 6, entry 8). GC results supported the observation of residual amount of A with CrLA1, CrLA2, and CrLA3 (7%, 15%, and 36%, resp., as shown in Tables 4, 5, and 6, entry 11). Hence, the optimization for the catalytic potential of CrLA1, CrLA2 or CrLA3 as a homogeneous catalyst for the oxidation of A to BA by an aqueous H2O2 is at 80 °C after 4 h with excellent conversion (93%, 85%, and 74%, for CrLA1, CrLA2, and CrLA3, resp.) and excellent chemoselectivity for all catalyst Cr(III)-complexes (100%) (Tables 4, 5, and 6, entry 11).
Unfortunately, after further time (6 h) at the same temperature, the chemoselectivity to BA was reduced and the conversion was improved awarding mixture of BA and BZ, with no deduction of other unknown side products (Tables 4, 5, and 6 of CrLA1, CrLA2, and CrLA3, resp., entry 12). After 6 h, the percentage of BA was highly reduced to be 75%, 69%, and 60% with improvement of BZ percentage to be 21%, 21%, and 9% (Tables 4, 5, and 6, entry 12) within CrLA1, CrLA2, and CrLA3, respectively.
Particularly, at 90 °C after 1 h (Tables 4, 5, and 6, entries from 13 and 16), the catalytic potential of CrLA1, CrLA2 or CrLA3 was excellent with low chemoselectivity to BA. The conversion was poor to award to 45%, 41%, and 37%, respectively. With further time (i.e., after 2 h, 4 h or 6 h) the control chemoselectivity reduced remarkably and the conversion increased as recorded from TON and TOF values in Tables 4, 5, and 6, entries 14, 15, and 16, respectively. This could be explained by the further oxidation of the desired product, BA, to BZ and other unknown and unwelcomed side products.
The same behavior was also observed at 100 °C for all catalyst complexes: CrLA1, CrLA2, and CrLA3. Low chemoselectivity and high uncontrol conversion are the results of the catalytic oxidation of R at 100 °C (Tables 4, 5, and 6, entries from 17 to 20). After 1 h, the yield of BA was good with presence of little amounts of BZ, unknown side products, and residual of the 13 reactants: 32%, 29%, and 26% of BA, 33%, 31%, and 28% of BZ, 10%, 7%, and 5% of other unknown side products, 25%, 33%, 41% of residual for CrLA1, CrLA2, and CrLA3, respectively (Tables 4, 5, and 6, entry 17). But by time, the amounts of the target product, BA, was decreased and, oppositely, the amount of BZ and the other unknown side products was increased as shown in Tables 4, 5, and 6 (entry 20) to reach at 6 h 15%, 13%, and 10% of BA, 60%, 57%, and 53% of BZ and 25%, 23%, and 19% of the other unknown side products for CrLA1, CrLA2, and CrLA3, respectively.
Figures 2, 3, and 4 show the conversion percentage of the benzyl alcohol oxidation by an aqueous H2O2 catalyzed by CrLA1, CrLA2 or CrLA3 at various temperatures and various times (Tables 4, 5, and 6 for CrLA1, CrLA2, and CrLA3, resp.). From Figures 2, 3, and 4, the highest uncontrolled conversion was at 100 °C affording 98%, 93%, and 82% of the reactant (R) oxidation using CrLA1, CrLA2, and CrLA3, respectively (Tables 4, 5, and 6, entry 20), with very low amount of the chemoselective product. On the other hand, at 80 °C, the oxidation process presents that the highest percentage of the chemoselectivity and the lowest of side products and reactant is awarded after 4 h (Tables 4, 5, and 6, entry 11) using CrLA1, CrLA2, and CrLA3; see TON and TOF values in Tables 4, 5, and 6. In particular, it could be concluded that the optimized oxidation process of benzyl alcohol to the target product BA by an aqueous H2O2 catalyzed by CrLA1, CrLA2 or CrLA3 is at 80 °C. Conclusively, all the above catalyst complexes have excellent catalytic activity for oxidation of benzyl alcohol under the optimized catalytic conditions to benzaldehyde.
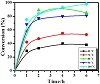
Figure 2: Effect of temperature and time on benzyl alcohol oxidation by H2O2 and catalyzed by CrLA1.
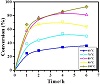
Figure 3: Effect of temperature and time on benzyl alcohol oxidation by H2O2 and catalyzed by CrLA2.
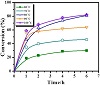
Figure 4: Effect of temperature and time on benzyl alcohol oxidation by H2O2 and catalyzed by CrLA3.
The solubility of the catalyst complex plays an essential role in the catalytic activity in a homogeneous system, as observed elsewhere [26,31,32]. Hence, the attached backbone coordinated ligand, polarity, and solubility of the current Cr(III)-catalysts may play a remarkable role for their catalytic potentials for the benzyl alcohol oxidation by H2O2 solution based on structure-activity relationship [33].
From the tentative Cr(III)-complex chemical structures (cf. Scheme 1 and [23]), the main difference in the chemical structure between CrLA1 and CrLA2 is the aromatic derivatives (i.e., o-ethoxy phenyl and naphthyl derivatives, resp.). It is already well known that naphthyl group is more organic in nature than the o-ethoxy phenyl group. Hence, CrLA1 has more nature than CrLA2 because of the naphthyl group and so it is more soluble in the oxidation process in acetonitrile as the organic solvent (acetonitrile). This suggestion increases the possibility that the oxidation reaction of benzyl alcohol catalyzed by CrLA1 is more homogeneous compared to that reaction catalyzed by CrLA2 [34]. This probability could be acceptable due to the solubility of CrLA1 and CrLA2 in acetonitrile. Moreover, the position of nitrate ion as a coordinated ligand in CrLA1 and as a counter ion in CrLA2, could allow the behavior of CrLA2 as a complex cation (Scheme 1) and reduces, particularly, its solubility in the organic solvent. So, the oxidation process could be more heterogeneous in nature in that solvent. This observation supports also the little higher catalytic activity of CrLA1 compared to CrLA2 in the oxidation process. The high observable difference between CrLA1 and CrLA2 in solubility and also in the catalytic potentials could be tough in the yield percentages of the chemoselective product for the oxidation of R to BA (93% and 85% of BA catalyzed by CrLA1 and CrLA2, resp.).
Generally, CrLA1 and CrLA2 are more reactive catalysts than CrLA3. To explain this behavior, the type of the coordinated ligands to the central metal ion, Cr(III) ion, may be the main reason. In CrLA3, the ligand behaves as a tetradentate chelate, while, in CrLA1 and CrLA2, the ligands are tridentate. Cr(III) ion forms octahedral geometric structures in CrLA1, CrLA2, and CrLA3 with coordination number 6; as shown in the tentative chemical structure of the studied complexes (Scheme 1). CrLA3 is more close and the ligand surrounds and capsulates Cr(III) ion to isolate it and afford more stable and less soluble complex (CrLA3) in polar organic solvents; whereas CrLA1 and CrLA2 are less stable than CrLA3 and more soluble in polar organic solvents (e.g., in methanol, acetone, and acetonitrile). Those reasons may be the main factors influencing the catalytic reactivity of the Cr(III)-imine complexes on the oxidation of benzyl alcohol to BA by an aqueous H2O2. Additionally, the presence of a labile coordinated solvent molecule in the catalyst complex (water as an open coordination site), as reported in many previous works [26,32], is playing an obvious role in the enhancement of the catalytic reactivity of the Cr-catalysts. This will be discussed in the mechanistic pathways. The presence of a labile coordinated water molecule in CrLA1, CrLA2 or CrLA3 may improve their catalytic activity (Scheme 2), but the steric demand of the coordinated imine ligands, as tridentate in CrLA1 and CrLA2 and as tetradentate in CrLA3, may play a major role in measuring the catalytic potentials of the studied Cr(III)-catalysts. The backbone ligand as more bulky with more steric demand influence in CrLA3 has less catalytic activity compared to CrLA1 and CrLA2 with the less bulkiness and steric demand. The main explanation is that studying of the mechanistic steps revealed that CrLA3, being more bulky and having more steric demand, reduced the availability of the substrate (R) or the oxidant (H2O2) to approach to the central metal ion, Cr(III) ion, in order to coordinate, forming a less stable active intermediate (see the reaction mechanism) [26]. On the other hand, CrLA1 and CrLA2 that are less bulky and have less steric demand are highly reactive towards oxidation of benzyl alcohol by an aqueous H2O2 for approaching and coordinating of the substrate (R) or oxidant with Cr(III) to proceed the oxidation process.
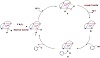
Scheme 2: Proposed mechanistic pathway for the oxidation of benzyl alcohol by an aqueous H2O2 solution catalyzed by Cr(III)-imine complexes.
Many transition metal complexes are considered as high effective catalysts for such redox processes. The strong impact of the nature of the involved organic or inorganic solvent could be observed in such benzyl alcohol oxidation processes recently [35,36,37]. The influence of various solvents with different organic nature (i.e., acetone, dichloromethane, chloroform, and DMF (N,N′-dimethyl
formamide)) on the oxidation of benzyl alcohol by H2O2 catalyzed by Cr(III)-imine complexes was investigated and summarized in Table 7.
Under the optimized reaction conditions of the highest active Cr(III)-catalyst complexes (CrLA1, CrLA2 or CrLA3), the effect of acetone, dichloromethane or chloroform was subjected and investigated. The results in Table 7 illustrate the percentages of the conversion control and chemoselectivity of R to BA. Particularly, the trend of the observed solvent effect was ordered as follows: acetonitrile > acetone > dichloromethane > chloroform > DMF for all Cr(III)-complex catalysts. In acetone, the control conversion percentages were good after acetonitrile (67%, 57%, and 51% of BA with CrLA1, CrLA2, and CrLA3, resp.) with unremarkable percentages of BZ. The oxidation in dichloromethane afforded moderate amount of the target product of BA using all Cr(III)-catalyst complexes with observable resulted amounts of other unknown side products (31%, 39%, and 31% using CrLA1, CrLA2, and CrLA3, resp.; cf. Table 7). In chloroform, the catalytic reactivity of all catalyst complexes was low with high uncontrolled conversion and with low chemoselectivity to BA. Particularly, the percentage of BA in chloroform is 25%, 24%, and 15%, using CrLA1, CrLA2, and CrLA3, respectively. Considerably, in DMF, there was on observation by GC for BA or BZ production in the oxidation process, the only products detected are unknown side products with very high percentages (cf. Table 7).
It is rationalized that the chemoselectivity of the catalytic oxidation of benzyl alcohol in those solvents is very poor with excellent uncontrolled conversion and unselectivity, compared to that in acetonitrile.
The polarity of the various solvents in the catalytic oxidation of alcohols may play an effective role for the chemoselectivity and conversion [26, 32]. In the highest polar solvent, acetone, the reactivity of all Cr(III) catalyst complexes CrLA1, CrLA2, and CrLA3, which afforded 67%, 57%, and 51% of BA, respectively, is overall good with detection of BZ and unknown side products. The catalytic potential of Cr(III) complexes is lower in acetone than in acetonitrile. Also, the conversion percentages of the oxidation process with all Cr(III) catalysts are the same percentages in acetonitrile, but the chemoselectivity in acetonitrile is higher than that in acetone (cf. Table 7). This may be attributed to the high solubility and polarity of CrLA1, CrLA2, and CrLA3, which progressed their catalytic potential but reduced their control and chemoselectivity. The effects of solubility and polarity of the Cr(III) catalysts could be realized in the less polar solvents, dichloromethane. The catalytic potential was very low with conversion in dichloromethane (53%, 40%, and 36% of BA) and chloroform (25%, 24%, and 15% of BA) with CrLA1, CrLA2, and CrLA3, respectively; see Table 7. The low solubility of the current catalysts may be the reason for their low reactivity in the less polar solvents (i.e., in chloroform and dichloromethane). Finally, in the high coordinated solvent, DMF, the studied complex catalysts are inactive in the chemoselectivity for the oxidation of R to BA; as reported previously [26].
It is assumed that in aprotic solvents (e.g., acetonitrile) the catalytic potential of CrLA1, CrLA2, and CrLA3 is highly chemoselective compared to the other solvents
(Tables 4, 5, and 6). It is also rationalized that the most effective solvent for the alcohol oxidation processes is acetonitrile; as reported elsewhere [11].
The effect of the catalyst was discovered by injection of different molar ratios of the catalysts (CrLA1, CrLA2, and CrLA3) in the benzyl alcohol in the oxidation process (0.02 mmol, 0.05 mmol or 0.10 mmol) using H2O2 in acetonitrile at 80 °C for 4 h (the optimized reaction conditions). In other words, the effect of the amounts of the catalysts is related to the amount of the substrate (benzyl alcohol) on the oxidation processes with 0.02 : 1, 0.05 : 1 and 0.10 : 1 (catalyst: benzyl alcohol). The results are reported in Table 8. The catalytic potentials of CrLA1, CrLA2, and CrLA3 at a catalytic amount of 0.02 mmol have been studied and reported in Tables 4, 5, and 6. The increase of the catalyst molar ratio to 0.05 mmol and 0.10 mmol caused improvement in the rate of benzyl alcohol oxidation
with higher conversion compared to the catalytic amount 0.02 mmol of the Cr(III)-imine complexes. Unfortunately, the chemoselectivity was reduced by increasing the amount of the catalyst Cr(III)-imine complexes to be 67%, 57.9%, and 61% of benzaldhyde with CrLA1, CrLA2, and CrLA3, respectively (at 0.05 mmol).
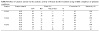
Table 8: Effect of catalyst amount on the catalytic activity of benzyl alcohol oxidation using Cr(III) complexes at optimum conditions.
When the catalytic amount of the catalyst increased to the double amount (0.10 mmol) of the catalyst complexes, their catalytic activity was more reduced to the chemoselective target product, as 45%, 44%, and 43.5% using CrLA1, CrLA2, and CrLA3, respectively (Table 8).
Although the increase of the catalyst complexes of the Cr(III) unit amounts (from 0.02 mmol to 0.05 mmol and 0.10 mmol) enhanced the reaction rate and the catalysts reactivity, it did not incubate the control chemo- and stereoselectivity of conversion. It increased the further benzyl alcohol oxidation to other unknown side products (Table 8).
It was observed that the presence of a labile coordinated solvent ligand in the complexes of CrIII (Scheme 2) may generate CrV (structure I) intermediate within electron transfer process due to the interaction of the oxidant with the catalyst complex as previously observed [26,38,39,40,41,42]. Particularly, the detection of Cr(V) intermediate species in the reactionmedia (structure II) has taken place by monitoring the repeated electronic spectral scans of complex in the oxidation process (Figure 5). The intensity of the characteristic maximum absorption bands are little shifted during the reaction. That little shift in the intensity of the characteristic absorption bands depends upon the addition of the oxidant to the catalyst complex in the reaction media. That little shift is probably due to the formation of active species in the oxidation reaction by the replacement of the coordinated labile solvent molecule (water molecule in structure I) with the oxidant molecule (i.e., H2O2 (structure II)) in order to form an oxide intermediate (structure II) within coordination of the oxidant to the central metal ion [43,44]. Several studies have been reported that the oxo-chromium(V) complexes are probably the intermediates in the oxidation processes of various organic compounds catalyzed by CrIII complexes [38,39,40,41,42]. Hence, the CrV=O active species are formed through the oxygen transfer mechanism from the coordinated oxidant molecule to give the active intermediate (structure III) with extraction of a water molecule [14]. The change in the color of the reaction in the beginning of the catalytic oxidation process initial solution from light green to brown highly supports the suggestion for the oxygen transfer from the oxidant (H2O2) to the central metal ion (CrV) to form oxo-chromium(V) species (structure III in the mechanistic proposal). When an aqueous H2O2 was added to the solution, the color slowly changed to brown. The observed change may be due to the generation of CrV=O species. This behavior expresses the interaction of peroxido group of the coordinated oxidant with CrV center ion and the presence of strong charge transfer transition in the new product (structure III). The active oxo-chromium(V) intermediate could oxidize the substrate (benzyl alcohol) through the coordination of benzyl alcohol to the active oxo-chromium(V) intermediate (structure IV) to regenerate the oxidized catalyst complex intermediate (structure I) and award the chemoselective product (i.e., benzaldehyde). Generally, the summarized mechanism of the catalytic processes for Cr3+-species is not enough clear [26]. However, on the basis of the color changes through the catalytic system of the reaction mixture and depending on the reported mechanisms for such reactions [38,39,40,41,42,45,46], it could be predicted here that the key step is the oxo-chromium(V) species formation in the catalytic process through the oxidation reaction of alcohols, as shown in Scheme 2, and as reported elsewhere [45,46].
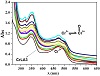
Figure 5: Repeated electronic spectral scan changes of CrLA3 catalyst with benzyl alcohol in the presence of an aqueous H2O2 in acetonitrile at 80 °C.